Our goal is to develop an aTF-based biosensor for HMA detection and increase the yield of HMA through the approach of directed evolution. Since PobR is an aTF naturally responds to 4-hydroxybenzoic acid, an analog of HMA, we employed the method of directed evolution to alter its selectivity and create novel aTFs responsive to HMA.
We built a PobR random mutagenesis library by error-prone PCR and MEGAWHOP PCR, and the generated library with highly random PobR mutations was transformed into E. coli DH5α. Then we used two reporters, eGFP and chloramphenicol resistance (Cmr) genes to screen for mutants that could respond to HMA. Then we further characterized the obtained mutants, and evaluated the contribution of each altered amino acid in the mutants to the ligand selection of the PobR protein. Finally, we obtained strains through adaptive laboratory evolution that showed up to 4.5-fold HMA yield.
Biosensor
ATFs-PobR
ATF-based biosensor can respond to a specific compound, or a series of compounds with similar structure, and can subsequently activate reporter gene expression, which have been leveraged as a tool for dynamic metabolic flux control, adaptive evolution, high-throughput screening and metabolite quantification [1-4]. However, there are no reports on aTF that can respond to HMA directly.
To our delight, HMA has a similar structure to 4HB, so we chose PobR that can respond to 4HB as our basic biosensor. Our strategy is that we select those strains that are able to produce much HMA by randomly mutating PobR to obtain PobRmut that responds to HMA specifically.
Expression vector
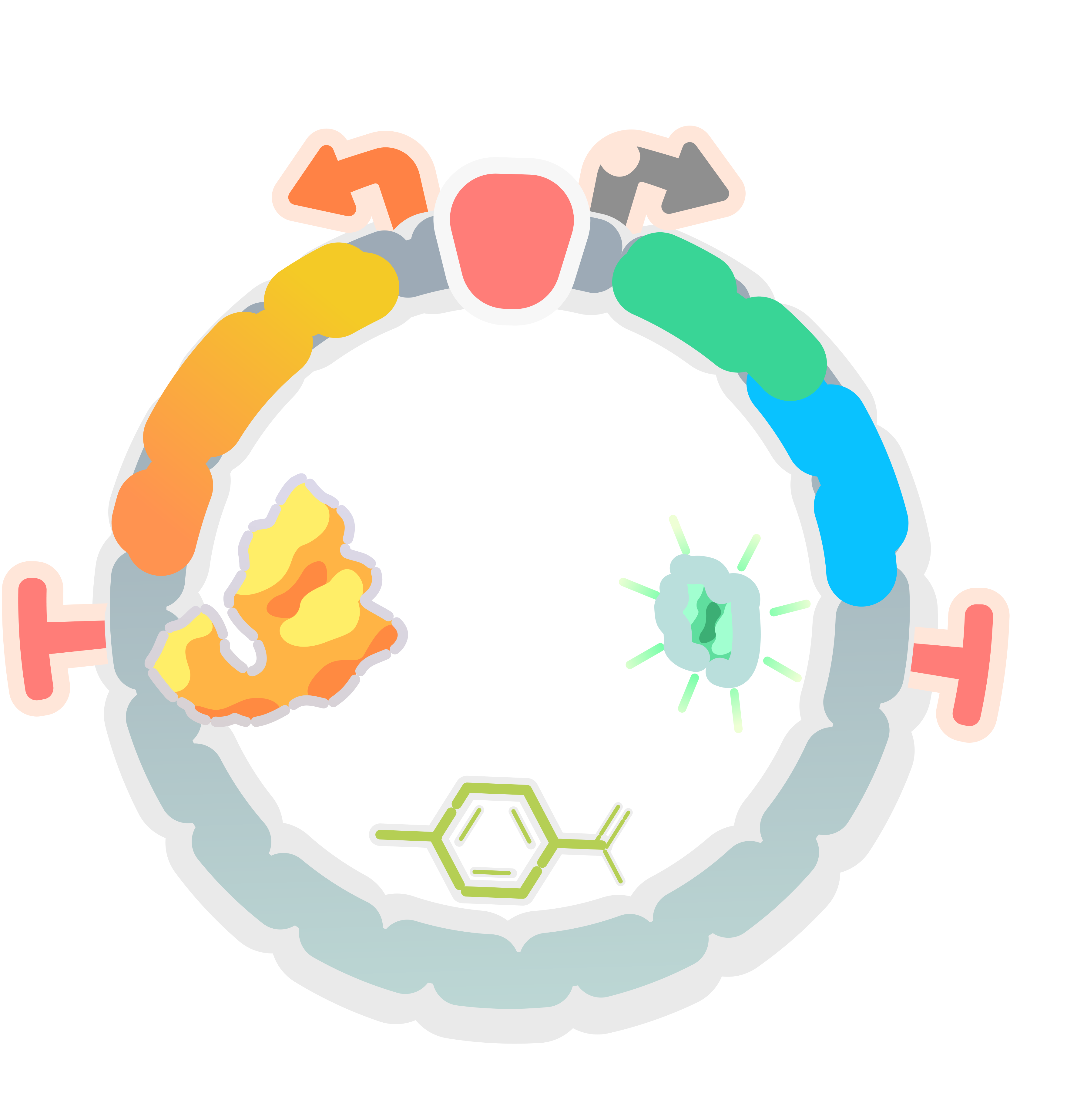
According to the codon preference to E. coli, we synthesized the sequence encoding PobR protein, and constructed the expression vector pYB1a-PobR-eGFP-Cmr.
In this expression vector, the PobR gene is driven by the constitutive promoter while the eGFP and Cmr resistance reporter genes are driven by the PpobA promoter. The consensus element (Oi) can be bound by PobR to inhibit the expression of reporter genes.
Selectivity
Characterization of ligand responses of wild type PobR
To evaluate the performance of the PobR biosensor, the plasmid named pYB1a-PobR-eGFP-Cmr carrying the PobRWT was transformed into E. coli, and the bacteria were cultured with increasing concentrations of 4HB.
To assess the specificity of the PobR biosensor in detecting different aromatic compounds, E. coli harboring the PobR biosensor is tested in LB medium supplemented by different molecules with similar structures to 4HB.
Design and construction of the PobR mutant library
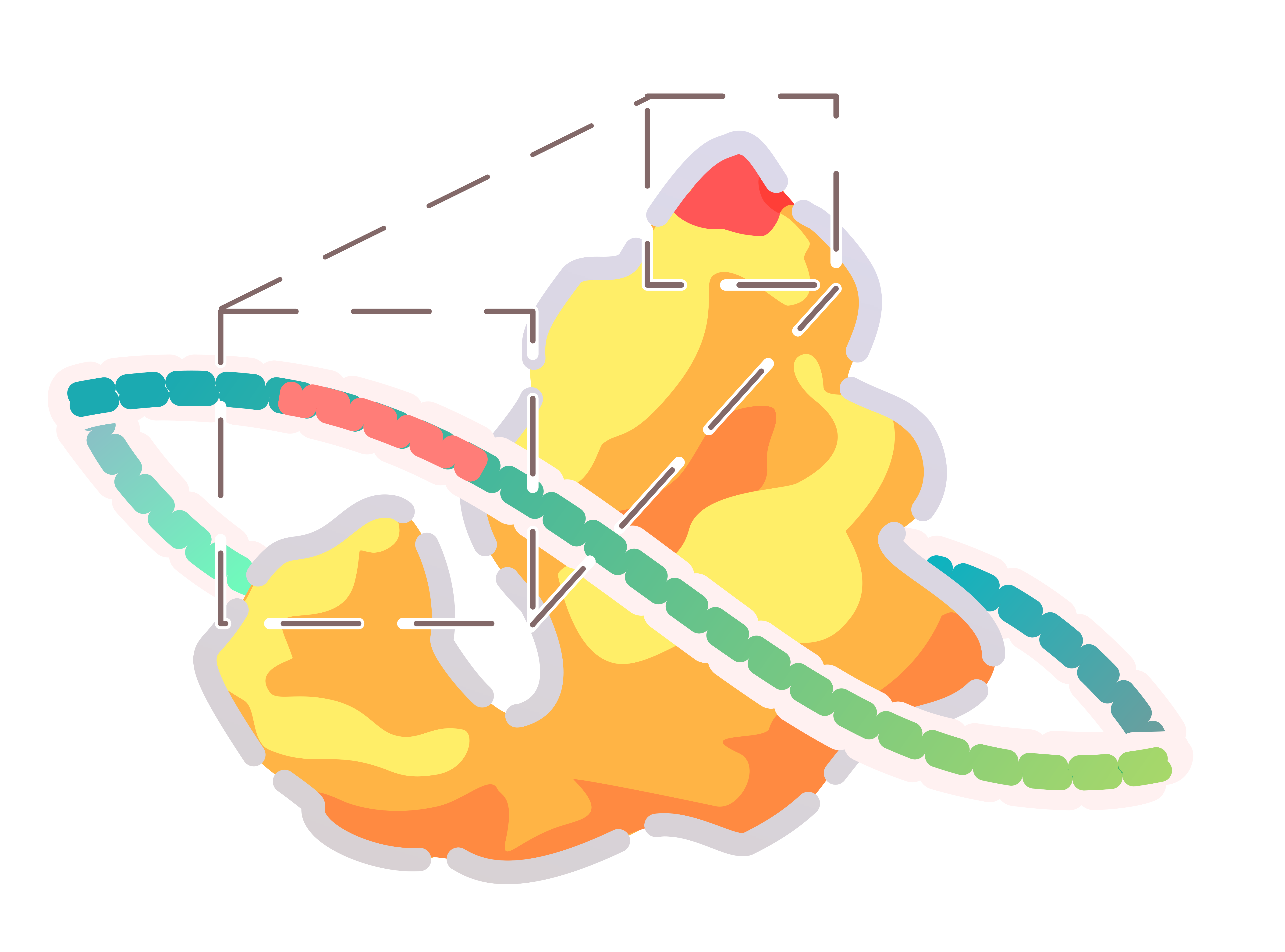
To generate various PobR mutants, we proceeded to develop a super large library through random mutagenesis of PobR using error-prone PCR and MEGAWHOP PCR amplifications.
Screening for HMA-responsive PobR mutants
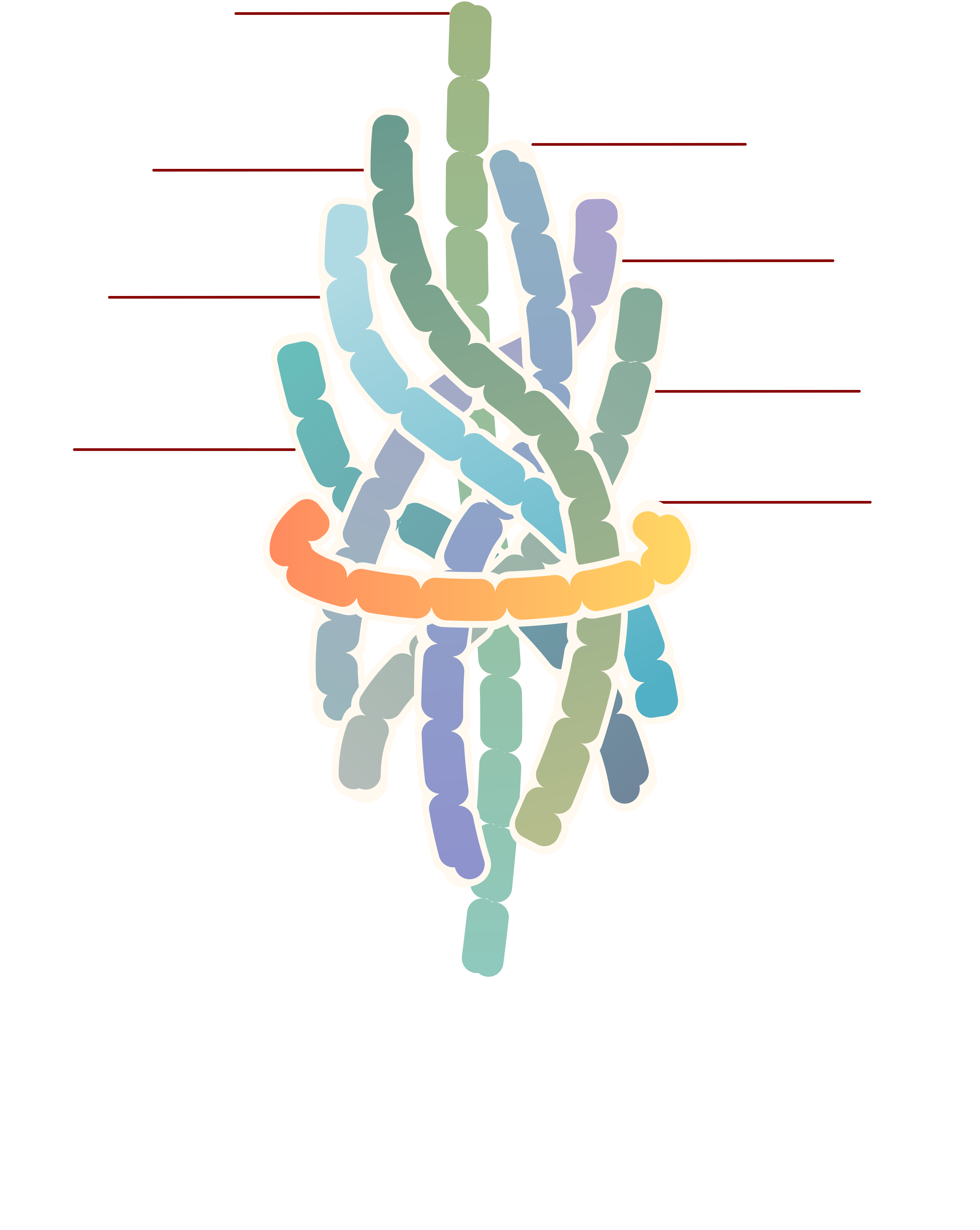
Antibiotic selection was first carried out to screen for HMA-responsive PobR mutants. The PpobA promoter regulates the expression of the Cmr gene. Therefore, if HMA could bind a PobR mutant and allosterically trigger its transcriptional activation of the promoter, the bacteria would survive on a plate media supplied with both chloramphenicol and HMA.
Coupling PobR transcriptional activity with chloramphenicol resistance could be used as a simple growth selection method to efficiently screen for HMA-responsive allosteric PobR mutants from our random mutagenesis library.
Testing
Ligand specificity and operational range of the two selected PobR mutants
To determine the response of PobR mutants to the ligands, bacteria carrying PobR plasmids were cultured in LB media with HMA concentration ranging from 0.01 g/L to 4 g/L.
To assess the specificity of the PobR mutants in detecting different aromatic compounds, E. coli harboring these PobR mutants were tested in LB medium supplemented by different molecules with similar structures to HMA.
Evaluation of the function of the identified amino acid mutations in PobR
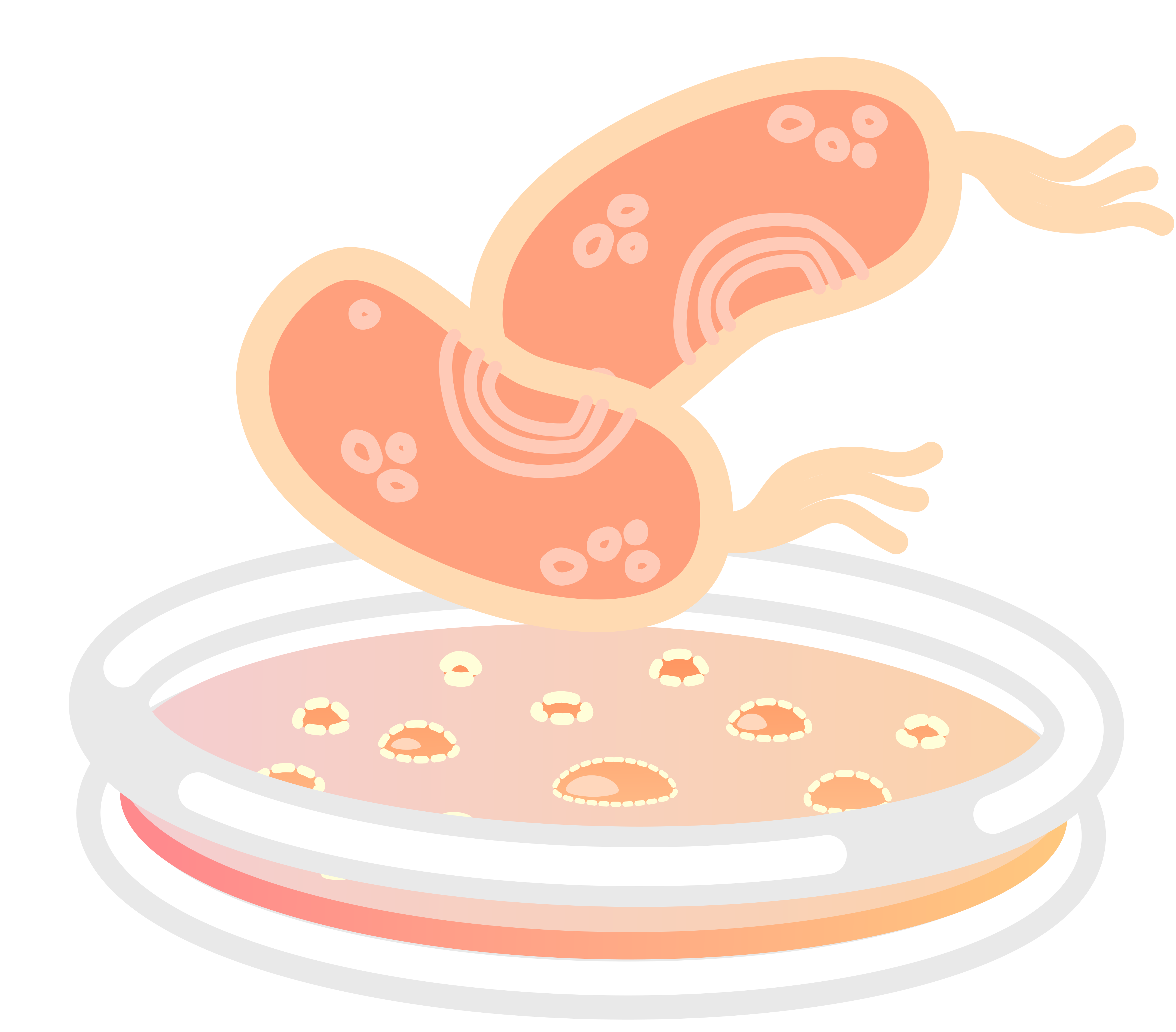
To determine how each amino acid among the mutated residues in the PobR protein contributed to the ligand selection, using site-directed mutagenesis, and conducted the experiments using our reporter system [5]. We expect to find out the reasons for the changes in adaptation and specificity of PobR protein pocket.
Modeling and docking
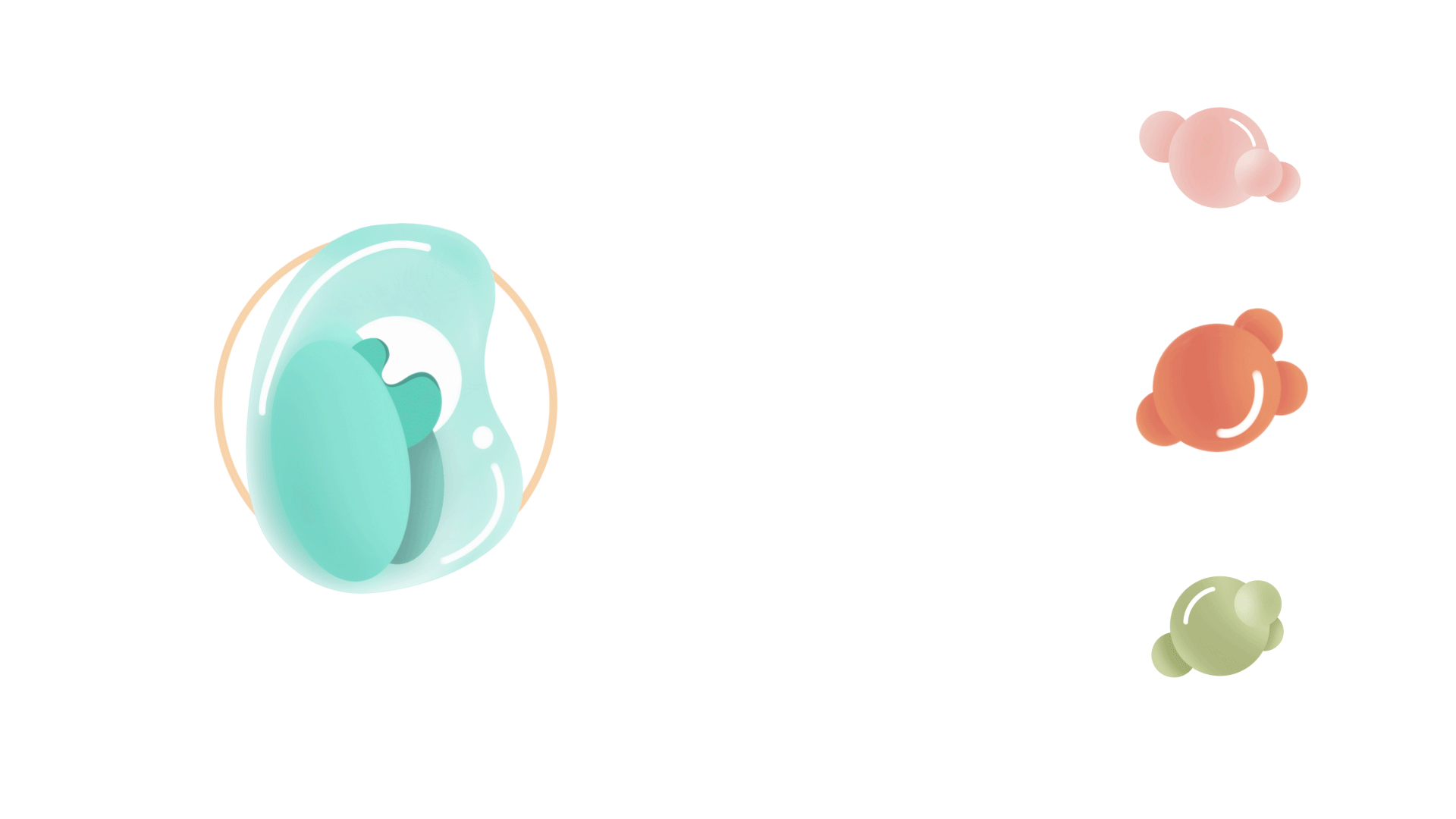
To more visualize the effects of amino acid substitutions on the response of PobR protein and the interaction between small molecule ligands and the protein receptor, we used AlphaFold2 to simulate the protein structure of the PobR mutant and Autodock Vina to simulate the docking of 4HB and HMA to the protein. The results of the docking were visualized by LigPlus [6].
By using bioinformatics methods to do molecular docking, we can more accurately understand how changes in single or multiple amino acid sites, especially around the active sites, affect the PobR protein pocket, and further explore how to affect the binding activity.
Evolution
HMA-Responsive Sensor-Driven adaptive laboratory evolution (ALE)
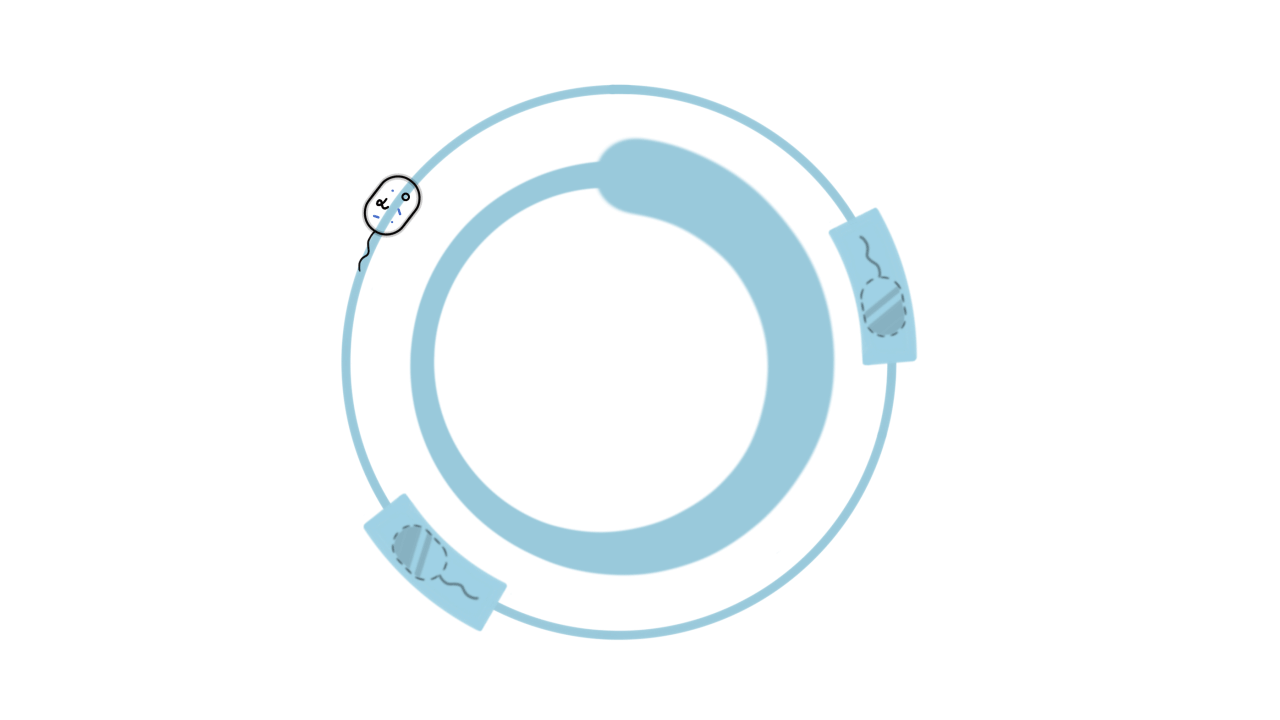
We hope to increase HMA yield through HMA-responsive sensor-driven adaptive laboratory evolution (ALE). To establish ALE, we coupled the HMA biosensor that we choosed with HMA synthase, 3-deoxy-7-phosphogentanoate synthase (aroG from E.coli ) and prephenate dehydratase (pheA from E. coli ), to construct an HMA biosynthesis module in BL21 (DE3). Six genes for scrambled proofreading, base selection and mismatch repair were also co-expressed in order to enhance the mutation of host genome replication [7]. This strain expresses chloramphenicol resistance only in the presence of HMA.
After that, we will select the appropriate concentration of chloramphenicol as the initial concentration, and then apply gradient pressure to the appropriate concentration of chloramphenicol. Finally, we will measure the production of engineered bacteria by High Performance Liquid Chromatography to verify our prediction.
References
- Mitchler MM, Garcia JM, Montero NE and Williams GJ. Transcription factor-based biosensors: a molecular-guided approach for natural product engineering. Curr Opin Biotechnol, 2021, 69:172-181.
- Gwon DA, Seok JY, Jung GY and Lee JW. Biosensor-Assisted Adaptive Laboratory Evolution for Violacein Production. Int J Mol Sci, 2021, 22:6594.
- Kasey CM, Zerrad M, Li Y, Cropp TA and Williams GJ. Development of Transcription Factor-Based Designer Macrolide Biosensors for Metabolic Engineering and Synthetic Biology. ACS Synth Biol, 2018, 7:227-239.
- Cheng F, Tang XL and Kardashliev T. Transcription Factor-Based Biosensors in High-Throughput Screening: Advances and Applications. Biotechnol J, 2018, 13:e1700648.
- Ogawa Y, Katsuyama Y, Ueno K and Ohnishi Y. Switching the Ligand Specificity of the Biosensor XylS from meta to para-Toluic Acid through Directed Evolution Exploiting a Dual Selection System. ACS Synth Biol, 2019, 8:2679-2689.
- Laskowski RA and Swindells MB. LigPlot+: multiple ligand-protein interaction diagrams for drug discovery. J Chem Inf Model, 2011, 51:2778-2786.
- Badran, A.H. and D.R. Liu. Development of potent in vivo mutagenesis plasmids with broad mutational spectra. Nat Commun, 2015. 6: p. 8425.